Radio Communications Blackout
Introduction
At high enough flight velocities, the intense heating of the gas generates a plasma layer around the vehicle. The presence of free electrons in the flow field has been known to hinder the transmission and reception of radio-waves. Historically, this communications problem has been of subsidiary concern in hypersonic design as usually the more common re-entry vehicles will only undergo blackout for a relatively short timeframe, while problems such as those dealing with heat transfer take precedence. This challenge, however, is likely to be a more significant obstacle for hypersonic glide systems as a considerable amount of the mission may lie within the blackout regime, potentially complicating guidance, tracking, radar identification, electronic countermeasures, and abort functionality. Although there are currently no proven blackout amelioration techniques, many possible solutions have been proposed and tested. Of all these techniques, both passive and active, the prevailing consensus over the past fifty years has been that vehicle/trajectory selection, electromagnetic manipulation, and material injection schemes are the solutions that have shown the most promise. It is fairly evident that there will likely be no universal elegant solution to the communications blackout problem. Instead, vehicle geometry and mission requirements will likely dictate a suitable mitigation strategy. The efficacy of two technologies (electromagnetic manipulation and material injection) are computationally investigated in the context of hypersonic cruise flight following the suggested communications design engineering approach for handling blackout (AFAL, 1970), by first evaluating if communications blackout can be avoided by antenna placement or higher frequency transmissions. When this is unachievable, then two leading technologies are evaluated based on primary design drivers, specifically size, weight, energy requirements, inherent changes to infrastructure (complexity), and use of already allocated frequency spectrum.
Mitigation Techniques
Trajectory and Vehicle Selection
There are numerous uncertainties associated with modeling the aerothermochemistry of a partially ionized hypersonic flow field, including the rates at which chemical reactions take place. The rates recommended by Park have been most widely utilized. Since these rates were determined empirically from experiments ranging between 300 K and 7000 K, there are inherent uncertainties present when extrapolating to higher temperatures. A detailed assessment of Park’s chemical kinetics model for use in communications blackout simulations is performed and compared with flight diagnostics of the RAM-C II flight.
Plasma profiles perpendicular to the RAM-C II wall are constructed and the maximum electron density is found from these lines for each axial reflectometer station location. A direct comparison between the maximum electron number density generated through trajectory point simulations and the reflectometer data is made to eliminate the assumptions inherent with interpolation. The reflectometer measurements have with horizontal bars depicting the altitude range in which the reflection coefficient experienced a definite rise. Comparisons are made between models using different number of species and different sets of forward reaction rate coefficients for the second reflectometer station, shown in the figure below. Detailed comparisons with all four reflectometers can be found in Ref. [1].
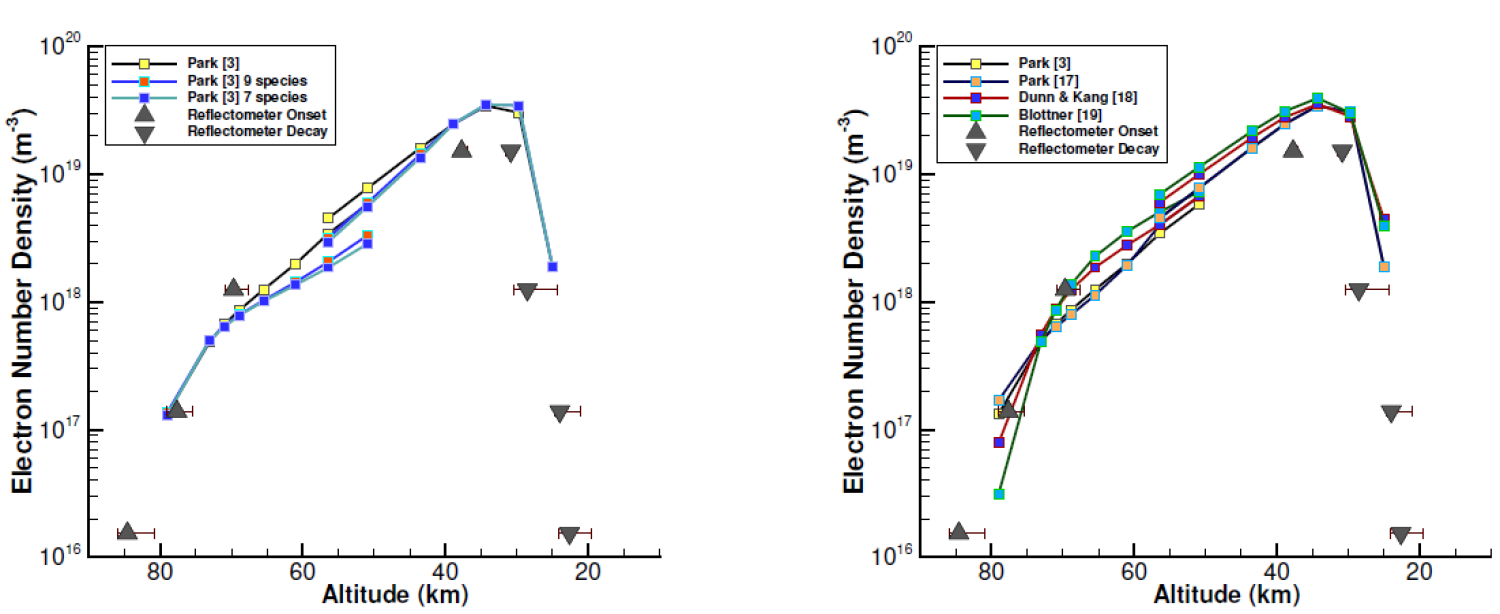
Direct comparisons of the maximum electron number densities calculated with the various equilibrium constants are made with the available electrostatic probe rake data (located 1.234 m downstream of the stagnation point). Both probes 1 and 8 are used here for comparison. The differences brought upon by the variation of the equilibrium constant models are displayed in the figure below and identify quantify the altitudes where recombination of electrons dictates the plasma flowfield. Whereas the electron densities calculated using Kim’s and Gordon & McBride’s equilibrium constants stay within 7% of each other throughout the trajectory, there is considerably more disparity between these models and that of Park’s, especially at low altitudes. In these low altitude regimes, the maximum temperatures near the electrostatic probe can be as low as 3,000 K. The differences of the equilibrium constants at low temperatures can play a significant role in electron concentrations.
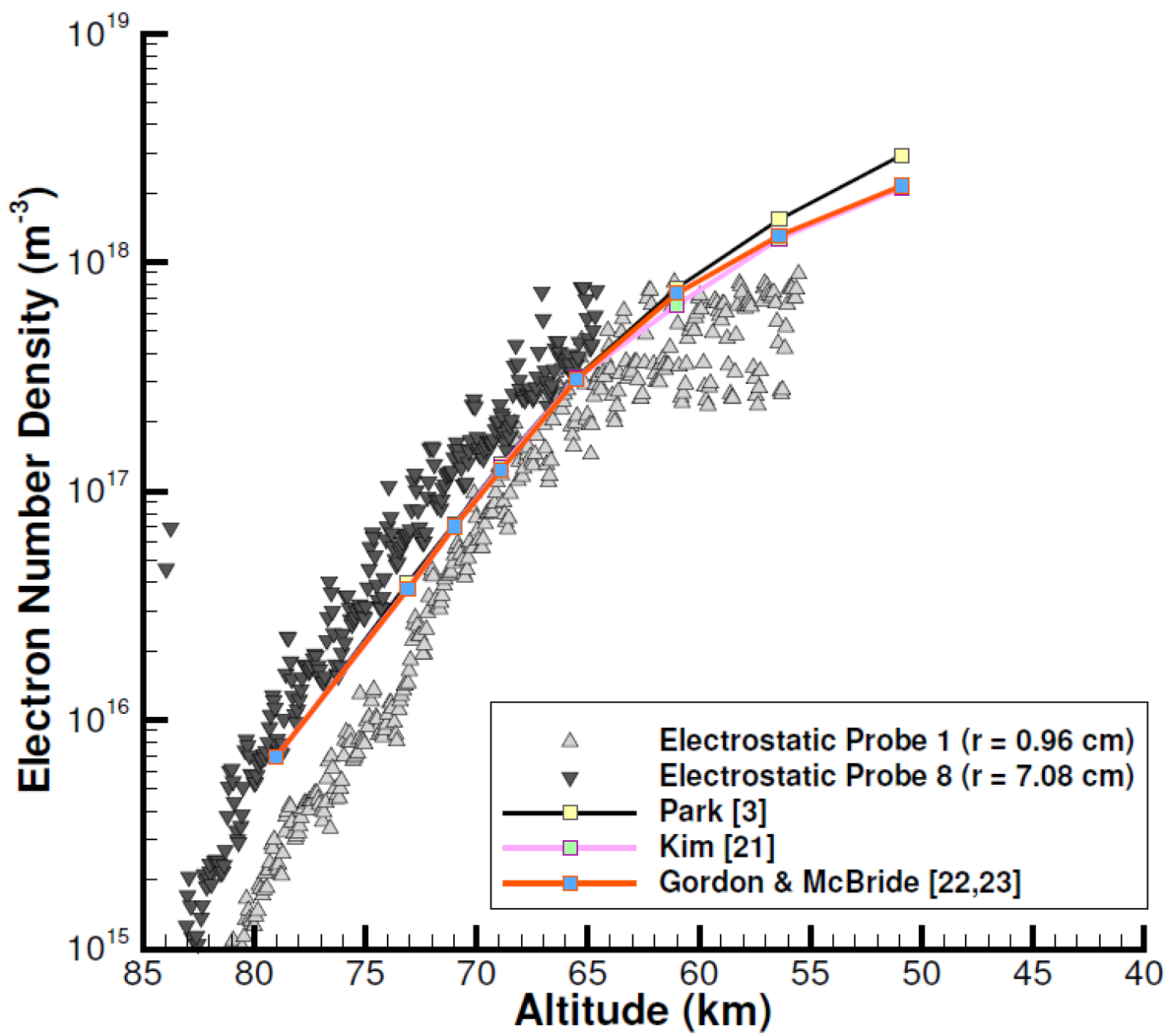
Comparisons between the inferred electron number density readings of stations 1 and 8 of the electrostatic probe rake and the
predictions obtained with different equilibrium constant models [1].
Electromagnetic Manipulation
The exploitation of electromagnetic fields as a blackout amelioration technique dates back to the early 1960s, when Hodara proposed that static magnetic fields could be used to create a window through which RF transmission can pass through will limited attenuation. By orienting the magnetic field lines such that the motion of the electrons is constrained through gyration, the dispersion relation becomes altered where a wave below the plasma frequency can propagate. The magnetic field limits the transverse motion of the electrons, and for an infinitely strong magnetic field, no interaction would take place between the traverse wave and electrons. This technique is one of the few to have been tested in flight, as signal attenuation and antenna impedance measurements were made during the RAM A-2 flight to determine the effectiveness of a magnetic window. Permanent magnets within the constraints of a hypersonic vehicle are limited to around 0.15 T and are hampered by the Curie temperature. With current technologies, a superconducting electromagnet would weigh over 500 kg and be difficult to implement. Nevertheless, many methods to reduce the magnetic field strength requirements and subsequently the system weight requirements have been proposed. For instance, the hybrid use of electrostatic fields has also shown promise, incorporating both magnetic window and electrostatic collection schemes. This system utilizes crossed electric and magnetic fields to collect both electrons and ions and accelerate the charged particles past the antenna, lowering the adjacent electron number density. This ExB drift technique has been demonstrated by Kim for use in blackout mitigation as seen in the figure below [2].
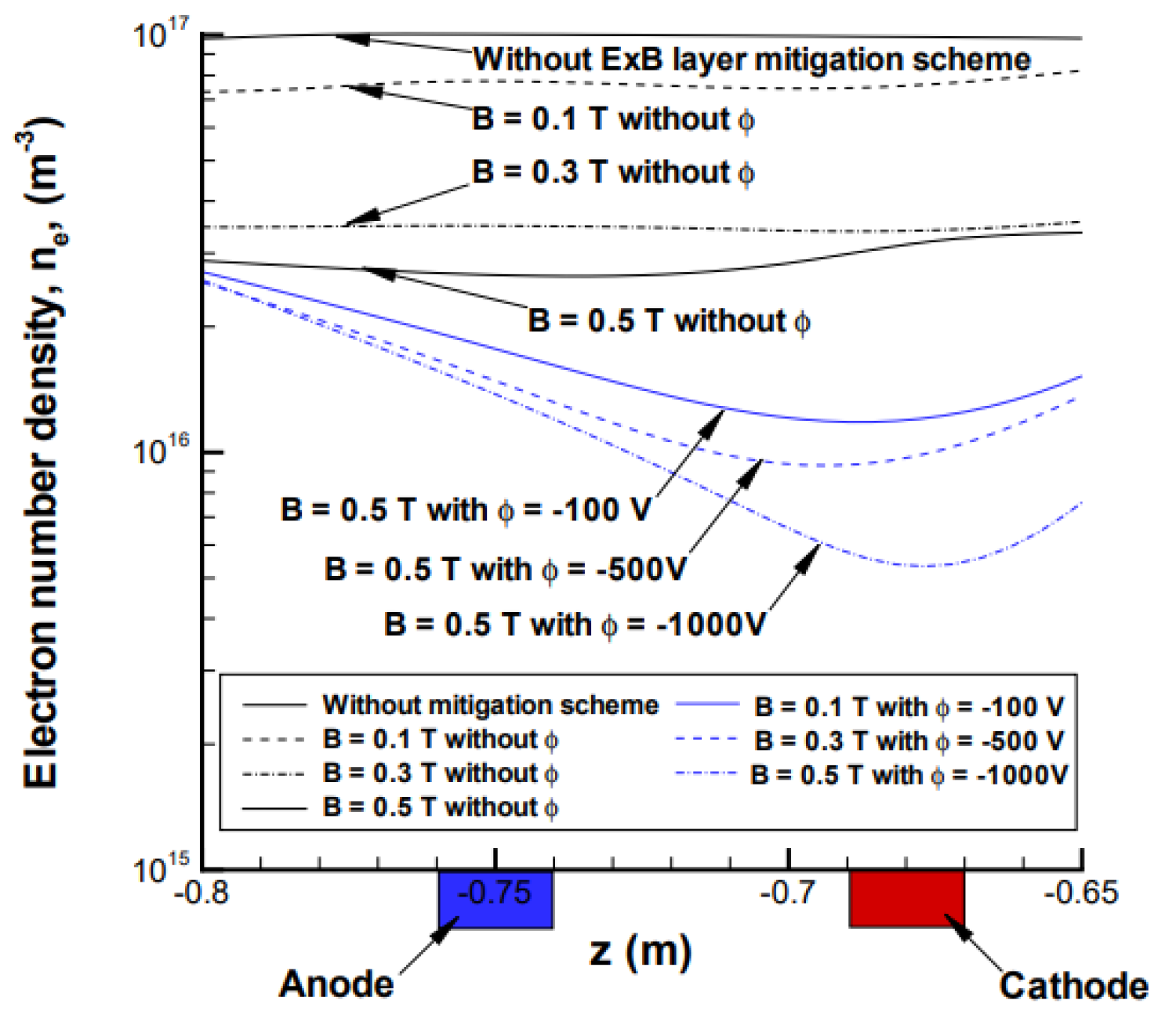
The electron number density distribution near the surface of OREX for several operational conditions of an E x B mitigation scheme[2].
Material Injection
Injection of material to the plasma flow upstream from the antenna has been consistently listed as one of the most promising techniques for alleviating the effect of the plasma layer. Among other factors, favorability to this mitigation technique can be largely attributed to its extensive testing in a number of flights, with several liquid injection experiments having been conducted during the RAM, Trailblazer, and Gemini flights, demonstrating varying levels of success. Injection of thermal quenchants lowers the temperature of the flow which in turn lowers the electron number density required to maintain equilibrium. As dissociating reactions are generally endothermic, chemicals with high heats of atomization are used as quenchants, such as water, propane, and freon variants.
As the water injection technique is one of the few with published flight data, it is beneficial to first validate the current numerical methodology. As such, comparisons with available flight data from the RAM-C I flight, specifically that of the electrostatic probe, are made, as shown in the figure below.
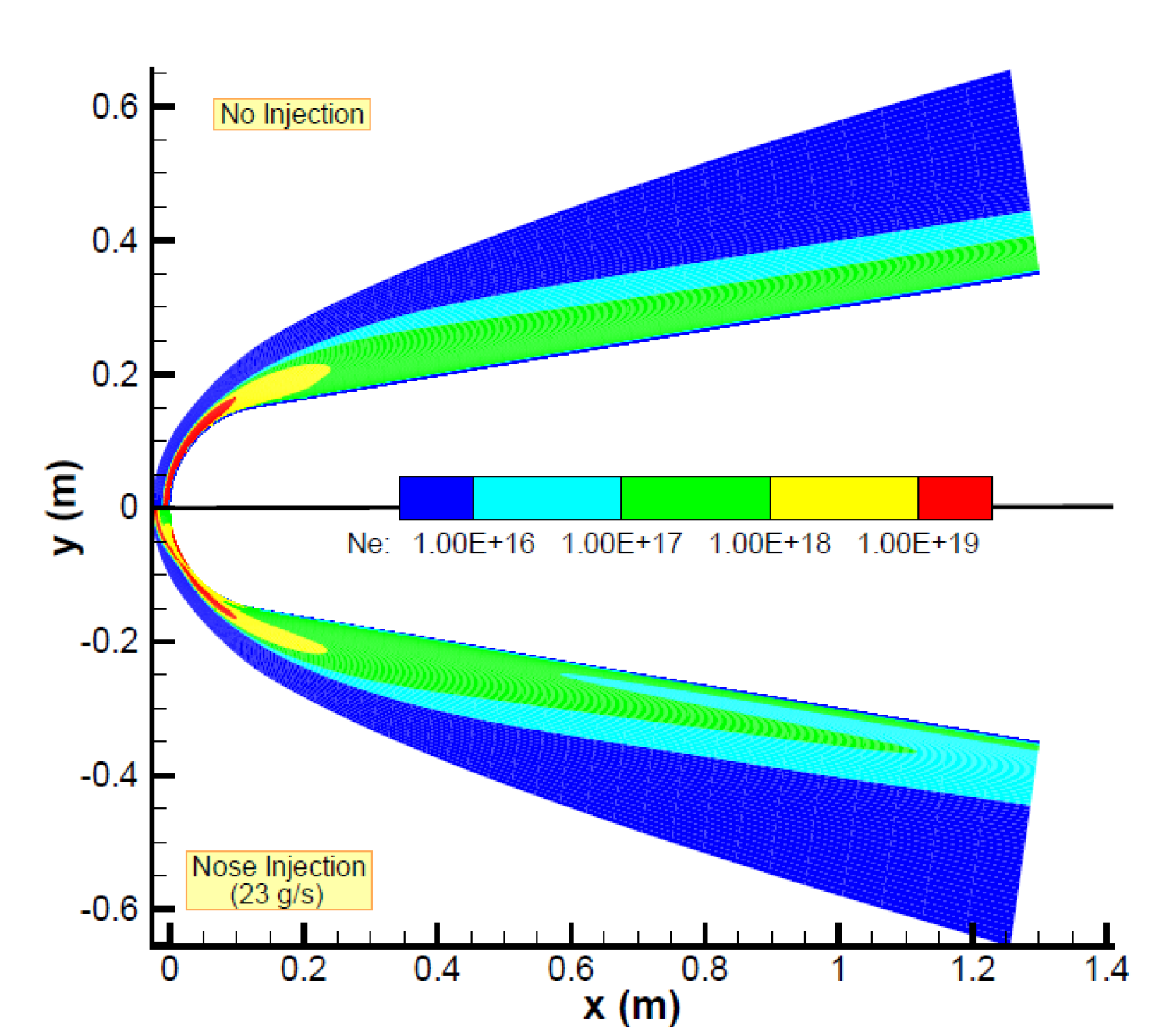
Electron number density of RAM-C I at 61km with no injection (top) and injection at the stagnation point; x = 0 cm (bottom).
Investigators
Pawel Sawicki
Acknowledgements
Funding for this work is provided by L3Harris Technologies.
References
[1] Sawicki, P., Chaudhry, R. S., and Boyd, I. D., "Influence of Chemical Kinetic Models on Plasma Generation in Hypersonic Flight", AIAA Journal, 2021
[2] Kim, M. K., Electromagnetic Manipulation of Plasma Layer for Re-Entry Blackout Mitigation, Ph.D. thesis, Department of Aerospace Engineering, University of Michigan, 2009.